Because the liquid content of many condensate reservoirs is a valuable and important part of the accumulation and because through retrograde condensation a large fraction of this liquid may be left in the reservoir at abandonment, the practice of lean gas cycling has been adopted in many condensate reservoirs. In gas cycling, the condensate liquid is removed from the produced (wet) gas, usually in a gasoline plant, and the residue, or dry gas, is returned to the reservoir through injection wells. The injected gas maintains reservoir pressure and retards retrograde condensation. At the same time, it drives the wet gas toward the producing wells. Because the removed liquids represent part of the wet gas volume, unless additional dry (makeup) gas is injected, reservoir pressure will decline slowly. At the conclusion of cycling (i.e., when the producing wells have been invaded by the dry gas), the reservoir is then pressure depleted (blown down) to recover the gas plus some of the remaining liquids from the portions not swept.
Although lean gas cycling appears to be an ideal solution to the retrograde condensate problem, a number of practical considerations make it less attractive. First, there is the deferred income from the sale of the gas, which may not be produced for 10 to 20 years. Second, cycling requires additional expenditures, usually some more wells, a gas compression and distribution system to the injection wells, and a liquid recovery plant. Third, it must be realized that even when reservoir pressure is maintained above the dew point, the liquid recovery by cycling may be considerably less than 100%.
Cycling recoveries can be broken down into three separate recovery factors, or efficiencies. When dry gas displaces wet gas within the pores of the reservoir rock, the microscopic displacement efficiency is in the range of 70% to 90%. Then, owing to the location and flow rates of the production and injection wells, there are areas of the reservoir that are not swept by dry gas at the time the producing wells have been invaded by dry gas, resulting in sweep efficiencies in the range of 50% to 90% (i.e., 50% to 90% of the initial pore volume is invaded by dry gas). Finally, many reservoirs are stratified in such a way that some stringers are much more permeable than others, so that the dry gas sweeps through them quite rapidly. Although considerable wet gas remains in the lower permeability (tighter) stringers, dry gas will have entered the producing wells in the more permeable stringers, eventually reducing the liquid content of the gas to the plant to an unprofitable level.
Now suppose a particular gas-condensate reservoir has a displacement efficiency of 80%, a sweep efficiency of 80%, and a permeability stratification factor of 80%. The product of these separate factors is given an overall condensate recovery by cycling of 51.2%. Under these conditions, cycling may not be particularly attractive because retrograde condensate losses by depletion performance seldom exceed 50%. However, during pressure depletion (blowdown) of the reservoir following cycling, some additional liquid may be recovered from both the swept and unswept portions of the reservoir. Also, liquid recoveries of propane and butane in gasoline plants are much higher than those from stage separation of low-temperature separation, which would be used if cycling was not adopted. From what has been said, it is evident that the question of whether to cycle involves many factors that must be carefully studied before a proper decision can be reached.
Cycling is also adopted in nonretrograde gas caps overlying oil zones, particularly when the oil is itself underlain by an active body of water. If the gas cap is produced concurrently with the oil, as the water drives the oil zone into the shrinking gas cap zone, unrecoverable oil remains not only in the original oil zone but also in that portion of the gas cap invaded by the oil. On the other hand, if the gas cap is cycled at essentially initial pressure, the active water drive displaces the oil into the producing oil wells with maximum recovery. In the meantime, some of the valuable liquids from the gas cap may be recovered by cycling. Additional benefits will accrue, of course, if the gas cap is retrograde. Even when water drive is absent, the concurrent depletion of the gas cap and the oil zone results in lowered oil recoveries, and increased oil recovery is produced by depleting the oil zone first and allowing the gas cap to expand and sweep through the oil zone.
When gas-condensate reservoirs are produced under an active water drive such that reservoir pressure declines very little below the initial pressure, there is little or no retrograde condensation, and the gas-oil ratio of the production remains substantially constant. The recovery is the same as in nonretrograde gas reservoirs under the same conditions and depends on (1) the initial connate water, Swi; (2) the residual gas saturation, Sgr, in the portion of the reservoir invaded by water; and (3) the fraction, F, of the initial reservoir volume invaded by water. The gas volume factor Bgi in ft3/SCF remains substantially constant because reservoir pressure does not decline, so the fractional recovery is

where Vi is the initial gross reservoir volume, Sgr is the residual gas saturation in the flooded area, Swi is the initial connate water saturation, and F is the fraction of the total volume invaded. Table 4.3 shows that residual gas saturations lie in range of 20% to 50% following water displacement. The fraction of the total volume invaded at any time or at abandonment depends primarily on well location and the effect of permeability stratification in edgewater drives and well spacing and the degree of water coning in bottomwater drives.
Table 5.7 shows the recovery factors calculated from Eq. (5.4), assuming a reasonable range of values for the connate water, residual gas saturation, and the fractional invasion by water at abandonment. The recovery factors apply equally to gas and gas-condensate reservoirs because, under active water drive, there is no retrograde loss.
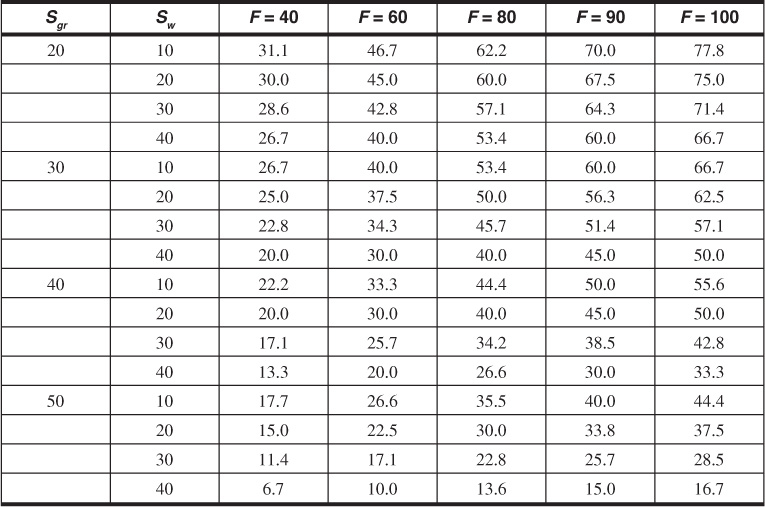
Table 5.7 Recovery Factors for Complete Water-Drive Reservoirs Based on Eq. (5.4)
Table 5.8 shows a comparison of gas-condensate recovery for the reservoir of Example 5.3 by (1) volumetric depletion, (2) water drive at initial pressure of 2960 psia, and (3) partial water drive where the pressure stabilizes at 2000 psia. The initial gross fluid, gas, and condensate, and the recoveries by depletion performance at an assumed abandonment pressure of 500 psia, are obtained from Example 5.3 and Tables 5.3 and 5.4. Under complete water drive, the recovery is 57.1% for a residual gas saturation of 20%, a connate water of 30%, and a fractional invasion of 80% at abandonment, as may be found by Eq. (5.4) or Table 5.7. Because there is no retrograde loss, this figure applies equally to the gross gas, gas, and condensate recovery.
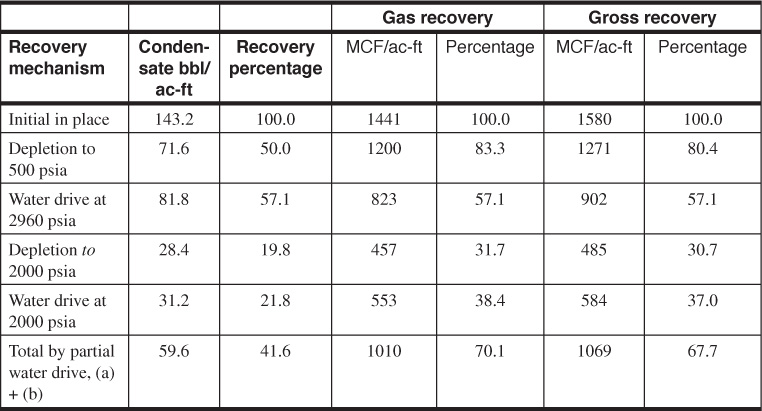
Table 5.8 Comparison of Gas-Condensate Recovery by Volumetric Performance, Complete Water Drive, and Partial Water Drive (Based on the Data of Tables 5.3 and 5.4 and Example 5.3. Sw = 30%; Sgr = Sor + Sgr = 20%; F = 80%)
When a partial water drive exists and the reservoir pressure stabilizes at some pressure, here 2000 psia, the recovery is approximately the sum of the recovery by pressure depletion down to the stabilization pressure, plus the recovery of the remaining fluid by complete water drive at the stabilization pressure. Because the retrograde liquid at the stabilization pressure is immobile, it is enveloped by the invading water, and the residual hydrocarbon saturation (gas plus retrograde liquid) is about the same as for gas alone, or 20% for this example. The recovery figures of Table 5.8 by depletion down to 2000 psia are obtained from Table 5.4. The additional recovery by water drive at 2000 psia may be explained using the figures of Table 5.9. At 2000 psia, the retrograde condensate volume is 625 ft3/ac-ft, or 8.2% of the initial hydrocarbon pore volume of 7623 ft3/ac-ft, 8.2% being found from the PVT data given in Table 5.3. If the residual hydrocarbon (both gas and condensate) saturation after water invasion is assumed to be 20%, as previously assumed for the residual gas saturation by complete water drive, the water volume after water drive is 80% of 10,890, or 8712 ft3/ac-ft. The remaining 20% (2178 ft3/ac-ft), assuming pressure stabilizes at 2000 psia, will consist of 625 ft3/ac-ft of condensate liquid and 1553 ft3/ac-ft of free gas. The reservoir vapor at 2000 psia prior to water drive is
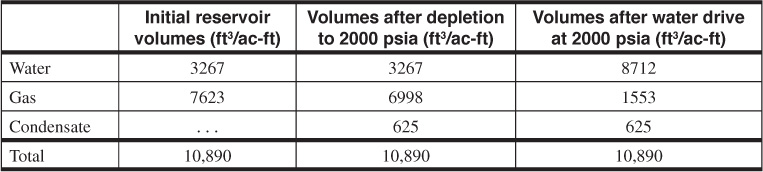
Table 5.9 Volumes of Water, Gas, and Condensate in 1 Acre-Foot of Bulk Rock for the Reservoir in Example 5.3

The fractional recovery of this vapor phase by complete water drive at 2000 psia is

If F = 0.80—or only 80% of each acre-foot, on the average, is invaded by water at abandonment—the overall recovery reduces to 0.80 × 0.778, or 62.2% of the vapor content at 2000 psia, or 584 M SCF/ac-ft. Table 5.4 indicates that, at 2000 psia, the ratio of gross gas to residue gas after separation is 245.2 to 232.3 and that the gas-oil ratio on a residue gas basis is 17,730 SCF/bbl. Thus 584 M SCF of gross gas contains residue gas in the amount of

and tank or surface condensate liquid in the amount of
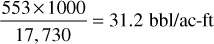
Table 5.8 indicates that for the gas-condensate reservoir of Example 5.3, using the assumed values for F and Sgr, best overall recovery is obtained by straight depletion performance. Best condensate recovery is by active water drive because no retrograde liquid forms. The value of the products obtained depends, of course, on the relative unit prices at which the gas and condensate are sold.
Leave a Reply