Many types of medical imaging techniques use quantum physics to reveal, diagnose, or examine disease in the human body. The first application came a mere two months after the German physicist Wilhelm Roentgen discovered the X-ray in 1895.
X-rays are produced when a beam of highly energetic electrons is directed onto a sold metal target. If the incident electron beam is energetic enough, it can liberate some of the metal atoms’ innermost electrons during collisions. Stability in the atom is restored when an outer electron makes a quantum jump to fill the hole in the innermost shell. When one of the outermost electrons drops all the way down to fill the hole, high-energy X-ray photons are emitted due to the large difference between energy levels.
Roentgen immediately realized that X-rays could penetrate low-density solid materials, including human tissue. In fact, he took the first X-ray picture of his wife’s hand to demonstrate the effect of this new type of radiation. Roentgen’s discovery earned him the first ever Nobel Prize in physics in 1901.
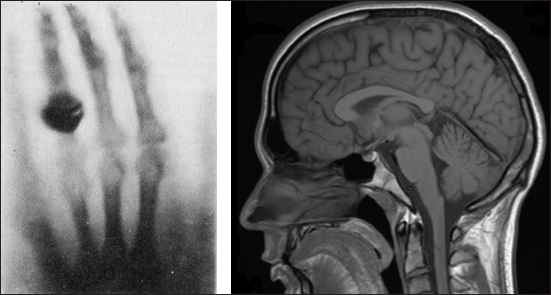
These images illustrate the improvements to medical imaging enabled by quantum physics. The image at left is Roentgen’s first X-ray image from 1895, a technique that indirectly applies quantum principles. The image at right is from a modern MRI, a technique that relies on quantum jumps and spin. (Right image courtesy of Helmut Januschka)
Max von Laue applied X-rays in 1912 to image crystal structure, and X-rays were used in 1952 to deduce the double-helix structure of DNA. X-rays have also been used to determine the structure of other biological molecules, such as insulin, cholesterols, hemoglobin, cells, and even some viruses, since all of these things can be crystallized.
Another important medical imaging technique makes use of quantum spin. Magnetic resonance imaging (MRI) detects the spin states of protons in the presence of two carefully controlled magnetic fields. It is particularly useful for medical imaging since the human body is composed mostly of water, which has many hydrogen atoms and therefore many “accessible” protons. The intensity of an MRI signal is directly proportional to water content, which allows for the production of high-resolution images of human tissues.
To take an MRI image, the patient is exposed to a pair of magnetic fields. The first of these is set at a constant value, and it acts to preferentially align the proton spin states in one direction, much like little compass needles. This happens because, in the presence of the static magnetic field, the “down” spin has less energy than the “up” spin by an amount equal to ΔE. More protons settle into the lower energy state than the higher energy state.
The device then applies a second magnetic field, which is oscillating at very high frequency (f). When the frequency of the field is such that f = ΔE⁄h, it is said to be in “resonance” with the spins. Then, photons from the oscillating field can be absorbed by spins states in the lower energy state when making an upward quantum jump to the higher energy state. Such a jump will absorb a photon from the oscillating field. This absorption of photons can be detected, and the more protons present, the higher the amount of absorption. The device can therefore quantify the amount of water present in the tissue. By carefully manipulating the two fields, a three-dimensional image of the tissue can be obtained.
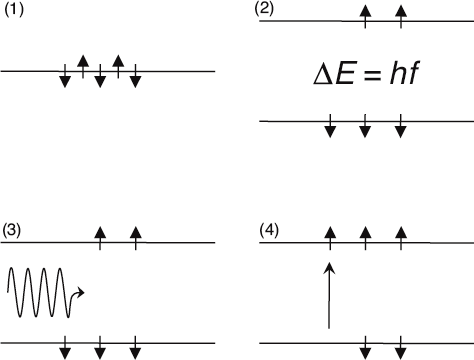
Here is a schematic illustration of MRI. With no magnetic fields present, all proton spins have the same energy (1). The constant magnetic field creates two distinct energy levels separated by ΔE = hf (2). When the dynamic magnetic field is at the resonance frequency f (3), a photon can be absorbed by one of the lower-energy spins (4). The amount of photon absorption is indicative of the density of the human tissue.
A final form of medical imaging we’d like to mention is positron emission tomography (also known as PET scans). The positron, as you’ll recall is the antimatter twin to the common electron. Positrons can be produced through the process of radioactive decay from unstable nuclei. They won’t last long when surrounded by ordinary matter, however, since they’ll quickly annihilate with electrons to produce high-energy gamma rays.
Positron emission tomography takes advantage of this fact to study the structure and activity of human organs. Prior to an image being taken, a radioactive, positron-emitting chemical is injected into the patient, who is then positioned within an array of photon detectors. These detect the annihilation gamma rays and can tell exactly where they came from. The data can then be processed to study the structure and function of the tissue from which the gamma rays emerged. PET scans have particular utility in monitoring brain function and disorders.
Leave a Reply